Where's the Synthetic Blood?

We are mostly blood. Of the 36 trillion cells in the human body, 32 trillion are blood cells. These blood cells synchronize the delivery of oxygen to every tissue in your body, fight pathogens, and heal wounds. However, they don’t last long; red blood cells circulate for just four months, and platelets for only ten days. To make a sufficient number of blood cells, your bone marrow acts as an extraordinary cellular factory, producing millions of blood cells every second to replace those that can no longer function properly. Under normal circumstances, replacement occurs quickly enough to replenish our supply. However, rapid blood loss due to trauma or surgery often necessitates a blood transfusion.
According to the American Red Cross, someone in the U.S. receives a blood transfusion or a blood product every two seconds. But currently, traditional blood transfusions face significant challenges, including a dwindling pool of donors and a limited shelf life for stored blood products. In January 2022, the American Red Cross declared its first-ever national blood crisis owing to a severe blood shortage—the worst shortfall in more than a decade. The shortage stemmed in part from COVID-19, which led to a 10 percent decline in the number of people donating blood compared to before the pandemic.
The creation of synthetic blood is one of the holy grails of biomedical research as it would alleviate such shortages and overcome current barriers to obtaining and storing blood products. There are a handful of efforts underway, but two overarching approaches to making synthetic blood products stand out: what I call the biologists’ approach and the chemists’ approach.
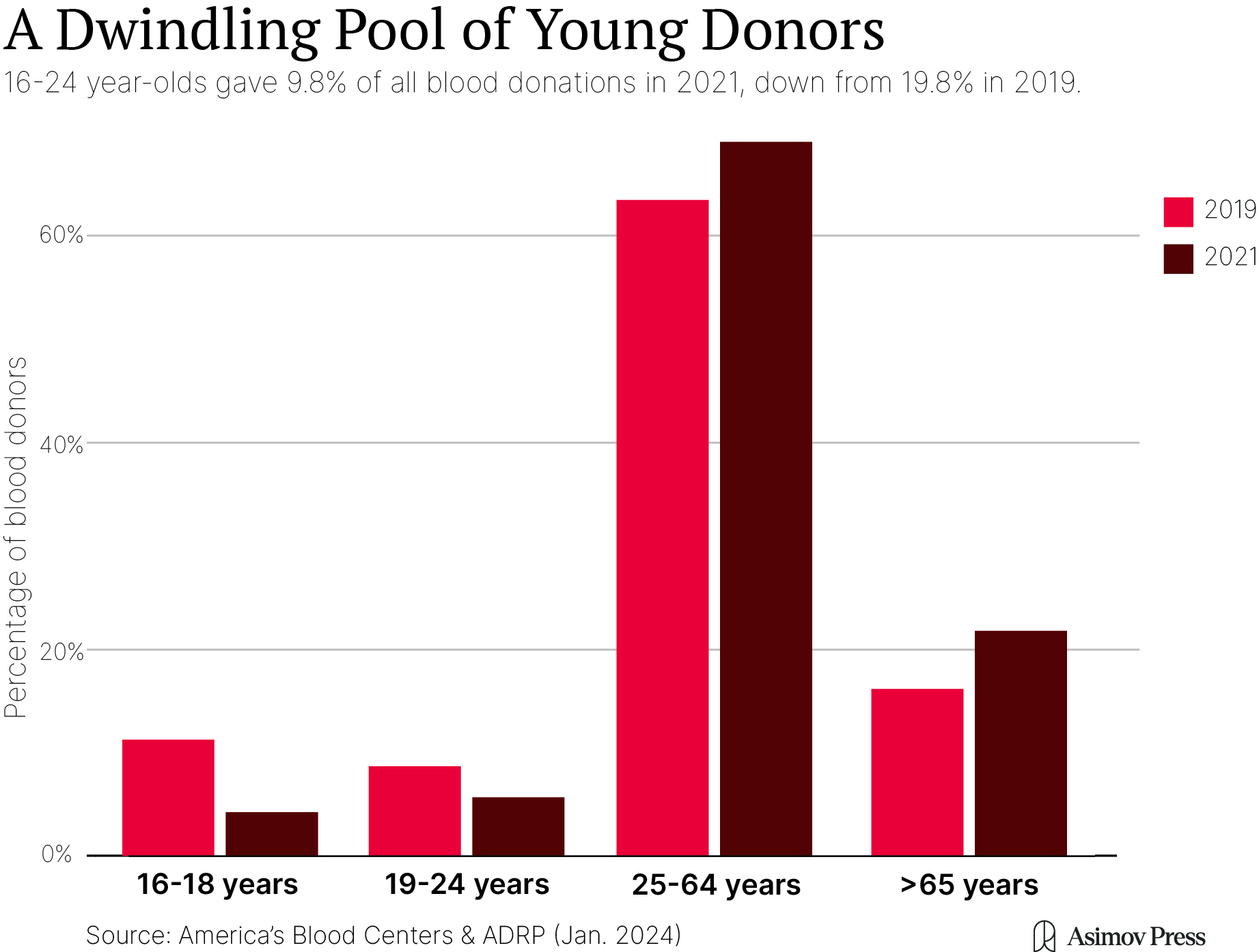
The biologists’ approach coaxes stem cells into becoming blood cells by growing them in bioreactors that recreate important features of the bone marrow, where blood cells are made in the body. The chemists’ approach synthesizes molecules and particles that mimic the functions of blood cells using methods suitable for large-scale manufacturing, thereby creating blood replacements that can be transported and stored more easily than cell-derived blood.
As a professor of bioengineering and hematology at the University of Colorado Anschutz Medical Center, I’ve seen the feasibility and efficacy of each approach demonstrated to varying degrees in the lab. However, developers of synthetic blood of either type will need to overcome economic and manufacturing challenges before they can scale for use in patients.
To better understand the challenges posed by traditional transfusions and the promising horizon of synthetic blood, we must first acknowledge that blood is more than the sum of its parts. A useful analogy is to think of a blood transfusion as you would an organ transplant. While blood doesn’t fit neatly into the various definitions of an organ, it still comprises a discrete system with specific functions. When a liver is transplanted, we don’t just transplant hepatocytes, the cells that are responsible for metabolism and detoxification, but also several other supporting cell types without which the liver couldn’t do its job. Similarly, blood has evolved as a system of cells suspended in a biochemically complex fluid with properties distinct from those of its individual components.
Clot formation is one example of blood’s complexity. As a clot forms, liquid blood becomes a solid plug that can stop bleeding in a matter of minutes. In the textbook description of clot formation, platelets aggregate to fill a hole in the blood vessel wall. The transfusion of platelets thus seems like an obvious treatment to stem bleeding. However, the process is actually a system-wide effort. Red blood cells push platelets toward the injured vessel while helping them activate to form a clot. And while platelets need the support of red blood cells to reach an injury site, they are far from passive, alerting white blood cells to contain any invading pathogens. Thus, all three types of blood cells work in concert to respond to breaches in the vascular wall; if any one of them is missing, the whole system stops working.
So whether we develop synthetic blood using the biologists’ or the chemists’ approach, we need to view blood as a highly integrated system. While this departs from the reductionist, single-component therapies that have dominated transfusion medicine since World War II, emerging data underscores that whole blood transfusions—blood with all its parts—yield better outcomes following severe blood loss than transfusions involving discrete blood components. However, it will become easier to understand why after we have taken a dip into the history of transfusion medicine.
{{signup}}
From Early Transfusion to Component Therapy
The earliest documented attempts at blood transfusions involved the transfer of blood from animals to humans in the 17thcentury. In a radical experiment in 1665, the English physician Richard Lower transfused blood from one dog to another. This experiment, performed before a rapt audience at the Royal Society in London, involved connecting the arteries of the two animals, and reviving the recipient dog from a state of lethargy as fresh blood flowed into its body.
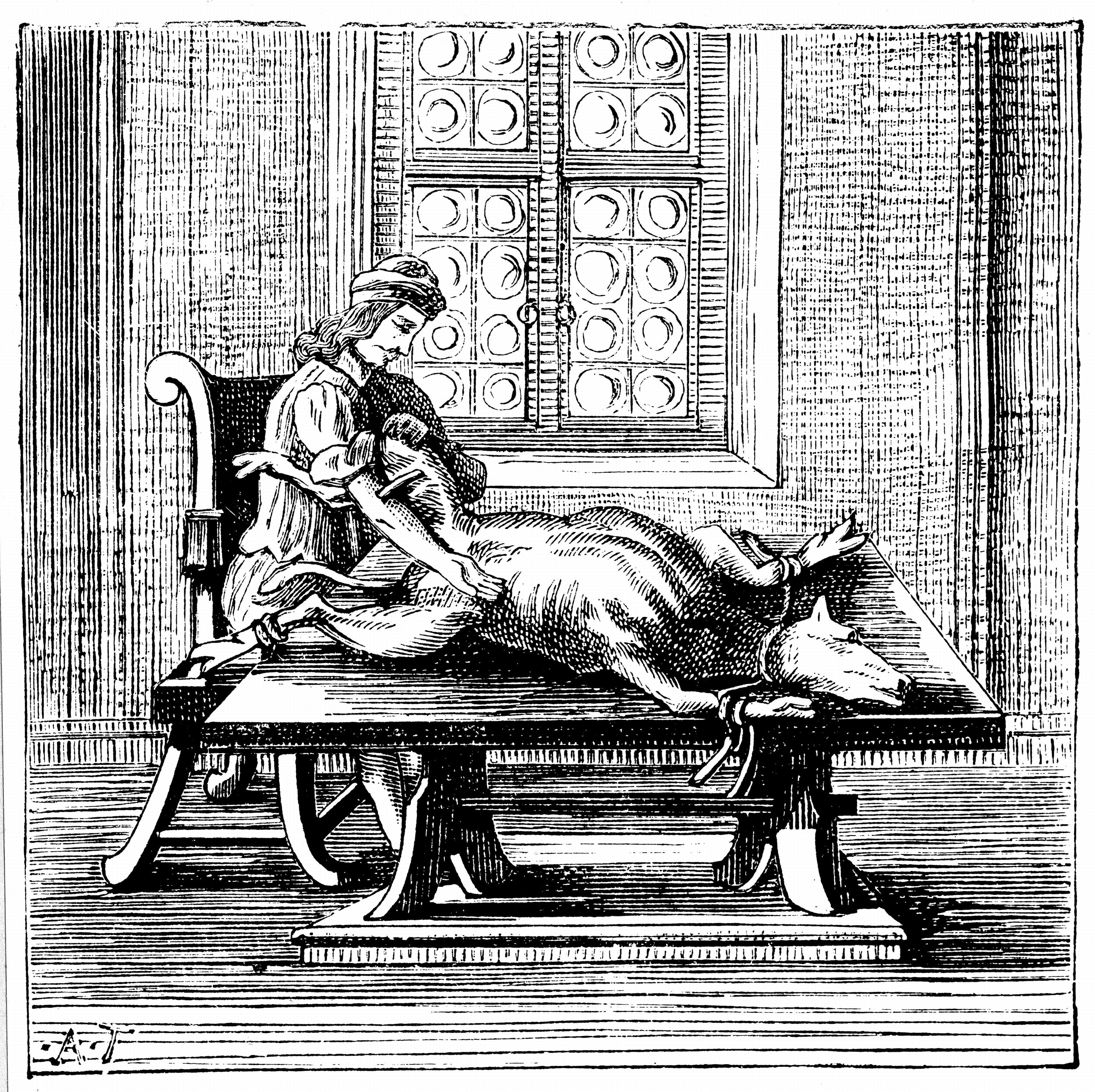
Meanwhile, in France, a physician named Jean-Baptiste Denys conducted more daring experiments in blood transfusion, this time from sheep to humans. He chose this animal subject due to the belief that the blood of a lamb could transfer its characteristics to human recipients, imbuing them with innocence and docility. This reflects the ancient Greek view that physical and moral traits could be transmitted through blood. Denys’ early successes involved a young boy and a robust porter, both of whom survived the procedure, with the porter reportedly exhibiting “renewed vigor” post-transfusion. However, the subsequent death of another patient thrust Denys into a contentious trial. Although he was acquitted due to evidence suggesting the patient died of arsenic poisoning at the hands of a spurned wife rather than from the transfusion itself, the incident, along with other transfusion-related deaths, led to a prohibition of the practice across Europe.
Over a century passed before physicians again dared human-to-human blood transfusions. In particular, blood transfusions were occasionally used following postpartum hemorrhage, but unpredictable allergic reactions impeded widespread adoption. The cause of these “allergic reactions” was elucidated by the Austrian-American biologist Karl Landsteiner's 1901 discovery of blood types, and marked a critical step toward understanding why some transfusions failed while others succeeded.1 Landsteiner observed that mixing blood from different people would sometimes result in clumps, indicating some sort of incompatibility. By categorizing these reactions, he identified the ABO blood group system.
While Landsteiner invented contemporary blood type matching, the transfusion methods of his day were markedly more primitive. Transfusions in the early 20th century were performed by directly connecting the donor to the recipient, a method that harked back to Lower’s dog experiments. The brutal trench warfare of World War I offered the first large-scale natural experiment in blood transfusions when it became imperative to move and share blood quickly. The Canadian physician Lawrence Bruce Robertson conducted 108 transfusions near battlefields in France for the British Royal Army, successfully resuscitating 75 percent of injured soldiers. At the same time, Oswald Robertson of the U.S. Army established the first blood banks in England and France, capitalizing on the recent discovery that sodium citrate could serve as a blood anticoagulant, allowing blood to be stored for several days. As stored blood was often needed expediently in response to traumas, these early military blood banks only carried blood from the universally accepted O group of donors to circumvent the need for blood-typing in urgent situations.

The wide geographic range of World War II precipitated a major bifurcation in blood transfusion and blood storage. Until then, almost all blood transfusions were of whole blood; blood with all its components as collected from a donor’s vein. However, refrigerated whole blood could only be stored for a few days, making its shipment from the U.S. to the European and Pacific fronts unfeasible. In 1940, Charles Drew—the first African-American researcher to earn a doctor of medical science degree at Columbia University—developed an ingenious method for separating and storing plasma, the liquid part of blood that contains essential proteins that promote clotting and regulate blood pressure. Drew also led the “Blood for Britain” project, collecting thousands of blood plasma donations in the United States to send to British soldiers on the frontlines. The isolation of plasma from whole blood set the stage for component therapy—taking blood apart and storing and transfusing its components separately.
Today, more than 100 million units of blood are collected worldwide annually, and component therapy is still the standard of care for most conditions needing blood transfusion. This remarkable capacity uses a similar process to that for the separation of milk into butter, cream, and skim milk. The raw material, in this case, whole blood, is extracted from a donor and then separated into its constituent parts. Each of these is stored under different conditions to optimize its shelf life. Plasma is frozen and good for up to a year. Red blood cells are kept in a refrigerator and can only be stored for a little longer than a month. Platelets are stored at room temperature for about a week as cold storage leads to their rapid clearance once in the body. Longer storage times for both platelets and red blood cells increase the risk of contamination by pathogens.
These three components—plasma, red blood cells, and platelets—are transfused individually or as a mixture, depending on the patient’s medical needs. Component therapy offers several advantages over whole blood. Plasma transfusions don’t need to be blood type matched and aid in managing acquired or congenital bleeding disorders. In other cases, only one type of blood cell needs to be replenished. Chemotherapy often causes low platelet counts, for example, and thus cancer patients only need to receive platelet transfusions. In contrast, people with sickle cell disease commonly receive red blood cell transfusions to alleviate vaso-occlusive pain crises. Component therapy allows more patients to be treated per unit of blood, with red blood cells going to one patient and plasma to another, reducing the overall demand for donations.

However, component therapy also has drawbacks. Even when plasma, platelets, and red blood cells are combined in a 1:1:1 ratio as a pseudo-whole blood, for example, the blood cells and plasma proteins are diluted by anticoagulants and media in storage. For this reason, a 1:1:1 ratio of these products may yield a volume fraction of red blood cells, or hematocrit, of just 29 percent compared to normal values of 35-50 percent. A related complication is “volume overload,” which occurs when the volume of blood components transfused exceeds a patient’s cardiovascular capacity to handle additional volume. This can cause fluid accumulation in the lungs, and thus low blood oxygen levels and a rapid heart rate as the heart works harder to pump the increased volume. In contrast, whole blood is either minimally diluted or not diluted at all.
While current transfusion practices and blood banking offer remarkable safety and reach, they also expose critical vulnerabilities. In the U.S., the blood supply relies on the altruism of an aging group of donors, with the baby boomer generation continuing to be the largest donor demographic. Gen X and younger generations don’t donate at nearly the rates that their parents and grandparents did at the same age.2 And while transfusions are among the safest procedures in medicine, there is still a very slight risk of transmission of infectious diseases and adverse immune reactions. In the U.S., where all blood donations are screened for infectious diseases, the risk of HIV or hepatitis C transmission is approximately 1 in 1.5 million units transfused. In low-income countries, blood donations are screened at lower rates and so the prevalence of transmitting infectious diseases (HIV, hepatitis B, and syphilis) is as high as 5 percent.
Finally, there remain significant disparities in access to safe blood transfusions, with low and middle-income countries facing more acute shortages.3 Of the 100 million units of blood collected each year, half comes from regions that account for just 20 percent of the world’s population, leaving an unmet need for another 100 million units each year. Driven by these shortfalls, the quest for synthetic blood aims to provide a sustainable alternative to the current reliance on blood donations.
Biologists’ Approach
Synthetic blood is a catch-all phrase for blood substitutes. This includes substitutes derived by culturing and differentiating stem cells into blood cells—the biologists’ approach. Or, alternatively, they refer to substitutes produced by assembling artificial cells that mimic blood cell functions or by modifying proteins like hemoglobin to act in a new or enhanced manner—the chemists’ approach.
The biologists’ approach to synthetic blood draws inspiration from how blood cells are made in the body. Blood cells derive from stem cells that are found primarily in the bone marrow. These stem cells differentiate into progenitor cells, which ultimately make the various types of blood cells in response to a series of chemical and mechanical cues, including feedback from the body telling them to slow down or speed up production.

It follows that the first step in the biologists’ approach is to find a source of raw materials, namely stem cells, to culture blood cells outside the body. The most readily available source of stem cells is—in a fittingly circular way—blood itself. It turns out that there are a small number of stem cells circulating in our bloodstream, comprising 1 million of the 32 trillion cells on the move. These circulating stem cells can be isolated, grown in flasks or steel tanks, and used to make synthetic blood products that can, in turn, be infused back into the person from whom the stem cells were derived. This is particularly beneficial for those who need chronic transfusions and are therefore at risk of developing an immune response to transfusion products from other people, or for those with religious beliefs, like Jehovah’s Witnesses, whose faith prohibits blood transfusions from another person.4
More than a decade ago, a Parisian research team reported the first transfusion of cultured red blood cells derived from circulating stem cells into a human. The cultured red blood cells had a similar function and circulation time compared to native red blood cells. Despite the importance of this achievement, though, deriving cultured blood cells from blood stem cells still requires that patients donate blood in the first place, a process that is woefully inefficient for most people other than the edge cases described above.
A better approach is to make “off-the-shelf” blood cells using pluripotent stem cells, or PSCs. These stem cells are made by reprogramming human cells, often from the skin, back into an embryonic-like state, allowing them to develop into any other cell type. Once established, PSC lines can divide and make blood cells indefinitely in culture, providing an inexhaustible source of blood without the need for donations.5
An added benefit of PSCs is that they are amenable to genetic modifications, making it possible to engineer blood cells with specific properties. For example, red blood cells have been derived from PSCs that are O-, the universally accepted blood type.

Making blood from stem cells involves two basic steps. First, the stem cells are proliferated into more stem cells. Then, the stem cells are differentiated into blood cells, which involves encouraging the stem cells to become specialized progenitor cells that ultimately become blood cells. Doing this efficiently requires simulating features of the bone marrow environment. This means presenting the growth factors, cytokines, and hormones in the right sequence in an environment that’s low in oxygen. However, simply adding the right cocktail of chemical cues doesn’t automatically lead to clinical quantities of blood cells. Blood progenitor cells need to be comfortable before they’ll make blood cells. And that comfort comes from familiar surroundings; a soft surface (not the hard bottom of a flask) surrounded by neighboring cells in a rush of flowing blood as progenitor cells release blood cells into circulation. To approximate these favorable surroundings, scientists have developed three-dimensional models that mimic features of the bone marrow.
In one example, researchers made platelets from progenitor cells, called megakaryocytes, in a scaffold made from silkworm cocoons that behave like bone marrow. At Harvard, researchers have crafted a microfluidic device in which megakaryocytes extend their platelet-releasing arms through small slits into a flowing stream that simulates the forces of blood flow. In each case, megakaryocyte cells in the laboratory only produced on the order of tens of platelets, far short of the 1,000 platelets generated per megakaryocyte in the human body. For this reason, it will be difficult to scale up stem cell-derived blood.
Cost is another challenge. There are two trillion red blood cells and 300 million platelets in each unit of blood transfused into the body. To reach this quantity with cultured blood cells, each stem cell needs to be expanded into thousands of new cells. However, this method quickly runs into some of the same economic challenges as another aspirational technology—lab-grown meat.

In both cases, the culture media containing the growth factors, hormones, and nutrients needed to grow cells can be expensive. Estimates vary, but one unit of cultured red blood cells costs $8,000-$15,000 compared to $250 for red blood cells provided by human donors. About 90 percent of that cost comes from the culture medium. There are promising ways to drive down the costs of the media, including recycling some of the most expensive components, using synthetic mimetics of hormones, and identifying new molecules to drive expansion, but this remains speculative.

Despite the high costs, researchers in Kyoto generated enough cultured platelets in 2022 to transfuse a 55-year-old woman for an initial human trial. The cultured platelets were made using a PSC-derived cell line of megakaryocytes in a turbulent flow bioreactor. Inside these tanks, blades move up and down to create turbulence that applies forces to suspended megakaryocytes, causing each one to produce nearly 100 platelets. The cultured platelets made in this bioreactor were well-tolerated by the patient, but still cleared from the body faster than normal platelets for reasons that remain unclear.
In the UK, RESTORE is a phase I clinical trial to assess the feasibility and safety of transfusing small amounts of cultured red blood cells (just one or two teaspoons) into healthy volunteers. The results of the trial are expected at the end of 2024. While the trial marks a promising start to testing synthetic blood in patients, the exorbitant costs of making lab-grown blood mean that larger clinical trials—an important stepping stone toward clinical use—may still be several years away.
Chemists’ Approach
The chemists’ approach to synthetic blood is to forego the complexities of cultured blood cells altogether and create artificial blood products. These artificial products are generally designed to do one thing and do it well, rather than replicate the cellular complexity that evolution has refined in whole blood over millions of years. The feature of blood that has stumped scientists the longest has been to find an alternative oxygen carrier to mimic red blood cells. Synthetic oxygen carriers are important in cases where someone has lost a lot of blood and urgently requires an infusion so that oxygen deprivation doesn’t damage the brain or heart.
As early as the 1960s, scientists turned to oxygen carriers like perfluorocarbons (PFCs)—chemicals that are also used as refrigerants. PFCs have a unique ability to dissolve and transport large quantities of oxygen; up to 20 times more oxygen than hemoglobin, the molecule that carries oxygen in the blood, on a per volume basis. Despite the initial promise of PFCs and FDA approval of a product called Fluosol-DA in 1989, PFCs have many drawbacks. Patients transfused with Fluosol-DA, for example, must breathe enriched oxygen during therapy. The chemicals also have a short shelf life and are toxic, which led to their eventual withdrawal from the market.
An alternative approach uses hemoglobin, the same protein red blood cells use to bind and carry oxygen through the body, as the backbone for what are called hemoglobin-based oxygen carriers (HBOCs). HBOCs are chemically modified human or animal hemoglobins that release oxygen in a way that mimics red blood cells even more faithfully than PFCs. Hemopure, the only FDA-approved HBOC product, is a bovine hemoglobin that is chemically crosslinked (that is, several hemoglobin molecules are bound to each other) to improve its stability. It is licensed in Russia and South Africa and is included in a few compassionate use programs across the globe, meaning it can be used clinically in cases where patients have few remaining options. However, clinical trials have shown that HBOCs can cause adverse cardiovascular events and kidney dysfunction, and they are quickly cleared from the bloodstream.
Many of the adverse events from trials of the first HBOCs were caused by hemoglobin crossing the blood vessel wall, where it scavenges nitric oxide and constricts blood vessels. One way to prevent this is to make the HBOC bigger by binding many hemoglobin molecules together or by decorating hemoglobin with long polymer chains. In a guinea pig model of hemorrhagic shock, “decorated” hemoglobin was able to resuscitate animals to the same degree as fresh, whole blood.
Another way to prevent HBOCs from crossing the vascular wall is to encapsulate hemoglobin in a particle, such as liposomes, to make an artificial red blood cell. Liposomes are synthesized from animal and synthetic lipids—the molecules that make up the membrane of cells. Each particle includes a lipid shell and a liquid core containing biomolecules. Dozens of FDA-approved therapeutics already rely on liposomes as drug-delivery vehicles including Doxil, the cancer drug, and Arikayce, which is inhaled and used to treat lung disease. Encapsulated hemoglobin circulates longer than free hemoglobin, especially when placed inside “stealth” liposomes that immune cells in the bloodstream don’t recognize as foreign substances.

For a 2021 study, rats were drained of 85 percent of their blood and then infused with liposome-encapsulated hemoglobin or red blood cells. Animals infused with the liposomes had similar survival and protection as those transfused with red blood cells against heart dysfunction, which is a complication associated with severe hemorrhagic shock.
A bevy of particles have been developed to replicate the functions of platelets in clot formation, which include adhering to the vessel wall, aggregating with each other, and pulling together to form an impermeable plug.6 SynthoPlateTM, for example, is a liposome decorated with peptides that allow it to help form clots by sticking to parts of an injured vessel wall and nearby platelets. When SynthoPlateTM was transfused into pigs following traumatic injury to the femoral artery, 80 percent survived compared to a control group that received no treatment, wherein none survived. Using a similar liposome platform that supports coagulation, researchers were able to stop blood loss from a mouse with low platelet counts to a similar degree as with a normal platelet transfusion.
The chemists’ approach draws upon a long history of drug delivery technologies, many of which have already gone through clinical trials and into use. Artificial blood made in this way is therefore cheaper to make and easier to manufacture than blood made using stem cells. Additionally, many of these artificial blood products, including SynthoPlateTM, have already been tested in animal models.
Getting Synthetic Blood in Circulation
The question of when these synthetic blood products will roll out and for whom remains unresolved—although we can make guesses based on clinical areas with the most need.
The transfusion of cultured blood cells using the biologists’ approach will likely first target people for whom donated blood product transfusions are problematic. This includes people with rare blood types that are hard to find a match for within blood bank reserves. For example, Rh-null, also known as Golden Blood, affects fewer than 50 people worldwide. Another vulnerable group is people who require regular transfusions, including patients with chronic anemias like sickle cell disease, because they can develop immune reactions against transfused blood products. Applications outside of transfusion medicine that do not rely on such large numbers of cells may be the first products to market. Platelets, for example, contain a multitude of molecules that play roles in wound healing and tissue regeneration. These can be extracted and used to promote healing in ocular surface disorders like dry eye syndrome. Cultured megakaryocytes or platelets could serve as a well-controlled, pathogen-free source of these products.
Artificial blood cells made using the chemists’ approach have a clearer path toward large-scale manufacturing, storage, and transfusions. After all, these are likely to use liposome formulations that have already been approved and are widely used for vaccine and drug delivery. Another key feature of artificial blood cells made in this way is that they can be freeze-dried into a powder, extending their shelf life for up to a year. Moreover, a powder doesn’t require refrigeration and has a much lower volume and weight than a liquid product, making these products well suited to under-resourced settings. Putting aside questions about storage and manufacture, the first application of artificial blood cells is likely as a bridge therapy during transport to the hospital rather than as a long-term blood substitute. That is, units of powdered artificial blood substitutes can be rapidly reconstituted into a liquid and injected into a hemorrhaging person to reduce bleeding and maintain organ oxygenation until they can receive a blood transfusion. This alone would be an enormous step forward, as half of trauma-related deaths occur before someone can even get to the hospital.
This is especially relevant in combat situations, which is why DARPA’s Fieldable Solutions for Hemorrhage with Autologous Resuscitative Products (FSHARP) program is spearheading initiatives to develop technologies on or near the battlefield, speeding up transfusions to wounded soldiers lacking access to human blood products. The program aims to synthesize a whole-blood mimic in response to two studies. One study showed that whole blood transfusion improved 30-day survival by 60 percent in people experiencing hemorrhagic shock, and a second that collected data on blood transfusions for soldiers in Iraq and Afghanistan confirmed that whole blood transfusions are superior to component therapies. A recent $46M award to a large consortium of researchers, including the inventors of SynthoPlateTM and ErthyroMerTM, a synthetic red blood cell, will determine in the next four years whether synthetic blood cells can achieve 90 percent of the functional outcomes of whole blood. The question of whether we will see synthetic blood soon is highly contingent on the success of such initiatives.
In the future, perhaps synthetic blood could perform even better than natural blood. Both the biologists’ and chemists’ approaches to synthetic blood and blood products have the potential not just to replace but to enhance blood function, creating bio-hybrid systems where engineered elements work in concert much like the advances seen in neural prosthetics.
Given that red blood cells have a long circulation time and come in contact with every organ and tissue in the body, synthetic red blood cells are also especially well-suited for diagnostics and drug delivery applications. Quince Therapeutics, a company in San Francisco, released a product called EryDexTM that uses a person’s own red blood cells to deliver the anti-inflammatory drug dexamethasone. It’s currently being explored in a clinical trial for a rare neurological childhood disorder, called ataxia-telangiectasia, which disrupts motor function and for which there are no available treatments. Cultured red blood cells can also be genetically engineered to act as synthetic sensors that detect circulating molecules inside the body. When a disease-related molecule binds to the sensor, for example, the engineered red blood cell could make a fluorescent signal that is later detected in a drop of blood.
Another emerging bio-hybrid system is microbots, which are small-scale robots designed to perform a specific task in the body. Rather than relying on blood flow to deliver these bots to their target, they are often directed by external magnetic or acoustic fields. One blood cell bio-hybrid microbot strategy is coating inorganic bots with blood cell membranes, thereby preventing them from being recognized as foreign materials by the immune system. This approach was used to develop an acoustic field-driven microbot coated with red blood cell and platelet membranes that could remove pathogens from the blood.
Ultimately, seeing synthetic blood within the next few years will ride on the success of the RESTORE trial, FSHARP, and related trials. It will also require bringing down the costs associated with culturing, and more insight into whether medical applications like microbots prove valuable. At a higher level, the future of synthetic blood awaits our ability to master and direct the symphony of interactions within blood. After all, synthetic blood is not just about replacing lost blood; it’s about revisioning what blood can do, turning a life-sustaining fluid into a dynamic and intelligent system that could address current shortcomings in transfusion medicine and improve patient outcomes in previously unimaginable ways.
{{divider}}
Keith Neeves, PhD is a Professor of Bioengineering and Pediatrics at the University of Colorado Denver | Anschutz Medical Campus. His laboratory develops technologies for the diagnosis and treatment of blood disorders.
Thanks to Saloni Dattani for proposing this essay idea and Joe Italiano and Anirban Sen Gupta for fruitful discussions.
Cite: Keith Neeves. “Where’s the Synthetic Blood?” Asimov Press (2024). DOI: https://doi.org/10.62211/92je-19ne
Footnotes
- In recent years, there has been remarkable progress in converting blood from one type to another. It’s now possible, for example, to convert A and B blood into O by using enzymes to modify antigens on the blood cells’ surface.
- The culture of non-remunerated blood donations started in WWII, when donating was considered a patriotic act, a motivation that may not resonate with younger donors. A 2020 Report to Congress from the U.S. Dept. of Health and Human Services recommends new studies on how to attract and retain a younger and more ethnically, racially, and geographically diverse blood donor pool.
- Some countries where this crisis is especially acute, such as Rwanda and Tanzania, have turned to unconventional blood delivery systems. Zipline, a San Francisco-based startup geared toward streamlining blood delivery, uses autonomous drones to transport blood from a distribution hub to the health care facilities. Today, they have made over 1,000,000 deliveries across these countries and more and have made significant strides towards preventing maternal deaths due to postpartum hemorrhaging.
- Chronic transfusions do not always put someone at higher risk of developing an immune response, but every donation from a new donor presents a different set of antigens to the recipient’s immune system. This means that, over time, a patient will develop antibodies against the common antigens that may present themselves with each new donation.
- There is a caveat in the linked studies, however. The Rafii lab’s paper was done with mouse cells, and the Daley lab’s method resulted in only a small fraction of red blood cells.
- There is no need to synthesize plasma because plasma is so easy to store. It can be frozen or freeze dried. “Synthetic plasmas,” transfused to regulate blood pressure and pH, are really just salt water (electrolytes) and maybe some albumin (to help with blood volume/pressure).
This article was published on July 7, 2024.
Always free. No ads. Richly storied.
Always free. No ads. Richly storied.
Always free. No ads. Richly storied.