The Mouse as a Microscope

It was 1905, and the French biologist Lucien Cuénot had a puzzle on his hands. He had been breeding mice in an attempt to decipher the patterns of coat color inheritance, but one of his crosses wasn’t behaving as expected. When Cuénot bred heterozygous yellow-coated mice—with yellow coloring as the dominant trait and black as the recessive—he observed two yellow mice born for every black one, instead of the predicted 3:1 ratio. It took another five years for a pair of American researchers to come up with an explanation before going on to pioneer the mouse as biomedicine’s premier model organism.
Cuénot’s puzzle seemed, at first, to be a violation of Mendel’s laws of inheritance. But such exceptions are typical of biology, wherein simple rules conspire together to produce incredible variation, obscuring our understanding like a dense fog. At that time, even though animal breeders had long exploited regularities in the patterns of inheritance, the principles underlying heredity remained mysterious. That is—until the Austrian monk Gregor Mendel showed that traits are passed from parent to offspring in discrete, independently assorted packages.
Mendel achieved this by measuring a small number of easily observable traits—such as seed color and shape—in a simple model system: pea plants. In general, the purpose of model systems, such as yeast, fruit flies, inbred mice, or Mendel’s peas, is to use a simpler representation to understand a broader or more complex phenomenon. Much like a microscope, a model organism is a lens that affects how we see the world—a narrow scope through which we derive a broader understanding. Cuénot’s experiments with mouse coats were one of the first attempts to transform the mouse into just such a microscope.
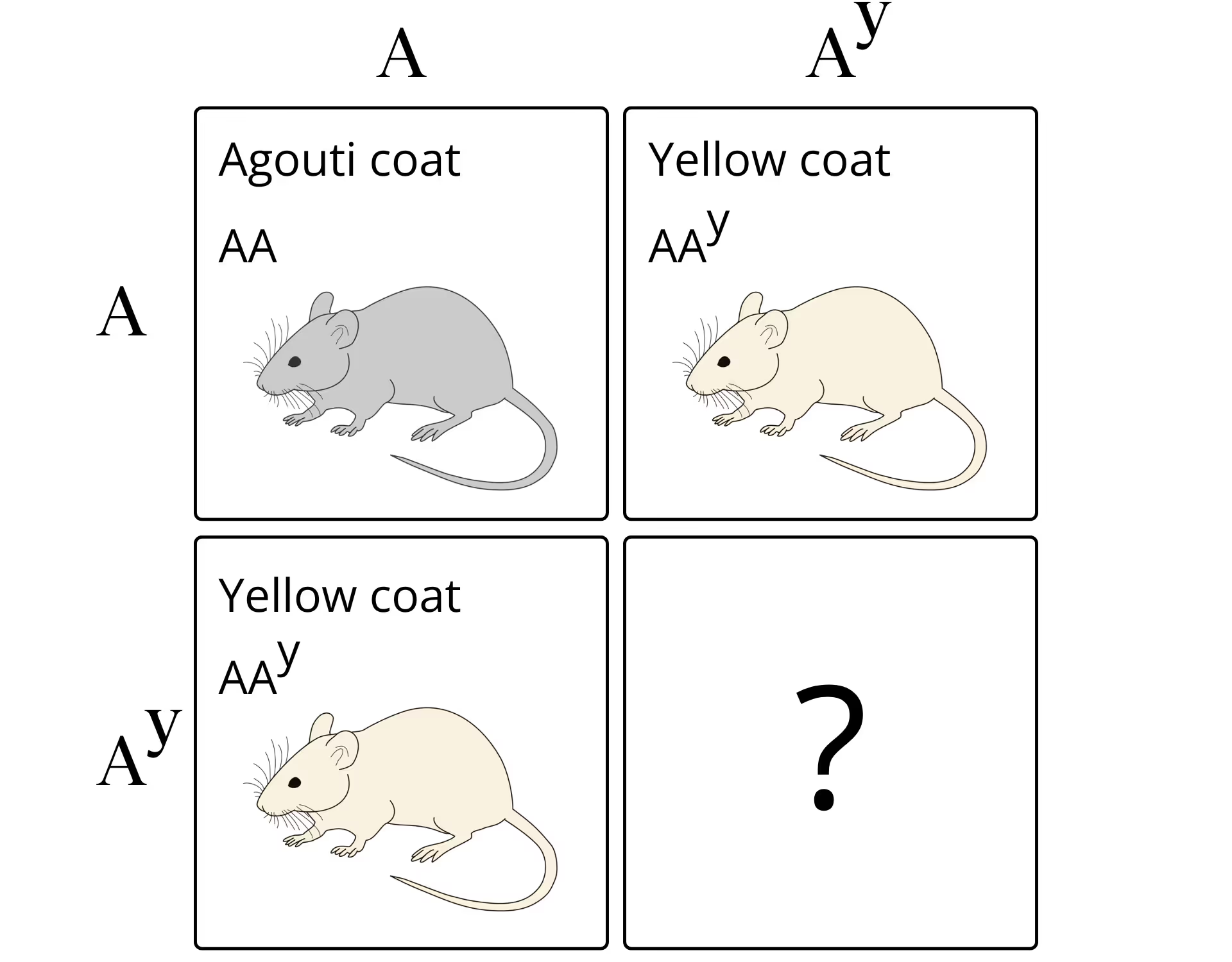
It took another century after Mendel’s pea experiments for the mouse to become an established presence in research labs. Along the way, experiments on mice enabled fundamental insights into the genetics and function of the immune system, as well as how to treat cancer with immunotherapy (along with many more discoveries too numerous to list). Without mouse models, we may never have developed polio and meningitis vaccines, organ transplants, GLP-1 drugs, gene therapies, or any other number of transformative treatments.
Today, somewhere on the order of 30 million rodents are used yearly in biomedical research in the U.S. and E.U. The majority of lab animals (about 95 percent) are mice or rats. However, the mouse’s modern prevalence was not guaranteed from the outset. The early supply of mice for research depended on a late-19th century community of hobbyists—fanciers—who collected, bred, and sold unusual mice varieties. These “fancy” varieties were then standardized in the 1920s by a small group of researchers at the Jackson Laboratory in Maine who intended to use them to investigate the genetics of cancer but were instead forced to sell their mice to support the lab when funding dried up during the Great Depression. From there, we witnessed an analog of the network effect, wherein the widespread sale and use of Jackson Lab mouse strains ensured their continued future use.
Standardization of mouse strains comes with benefits, but there are also risks with relying on such a small pantheon of models. Insights derived from one model organism can give us an incomplete and biased picture of the world; what proves true within one organism may not play out in another. This discordance, or lack of “predictive validity” when translating results across organismal boundaries, is perhaps most harmful in the biomedical field; despite extensive testing in mouse models, only 10 percent of drugs that make it to clinical trials ever make it to market. And while the inadequacies of model organisms are not solely to blame for this lackluster performance, it is fair to say that they bear substantial responsibility. After all, when everyone looks through the same lens it’s easy to miss the blind spots.
{{signup}}
A “Chemically Pure Animal”
While their ubiquity may be an accident of history, mice did not become the dominant animal model for biomedical research purely by chance: they have short generation times, large litters, and are small and relatively easy to handle. Mice, being mammals, are superficially (two eyes, two ears, four limbs) and genetically similar to humans; 99 percent of human genes have a counterpart in mice, and the protein-coding genes we share are 85 percent sequence-identical. This puts mice somewhere between pigs and primates in terms of sequence similarity with humans.
Mice also fall into an ethical goldilocks zone. They are similar enough to us to be scientifically useful, but dissimilar enough—partly owing to size bias and their history as pests—that we are not squeamish about using them for research in the same way that we are for cats, dogs, and monkeys. However, there's a shallower reason for the ascent of the mouse that probably dominates these post hoc justifications: mice, in both volume and variety, were historically easy for scientists to get hold of.

Mouse fanciers—hobbyists who collected and bred unusual varieties of mice—were prominent at the turn of the 20th century. These rodent enthusiasts were part of a broader flourishing of “animal fancy” whose practitioners dealt in dogs, cats, pigeons, and other charismatic animals. Animal fancying was a precursor to pet-keeping that emerged in the West during the Industrial Revolution, particularly in the Victorian era. Animal fancy, and later pets, filled a void left by the sequestration of animals away from urban settings into agricultural or wild domains.
Mouse fancying in particular probably originated in Asia, most likely China, and grew to popularity in the 1700s in Japan, where varieties named for their fur color—such as “Albino,” “Black,” “Chocolate,” “Champagne,” and “Lilac”—were prized. A particularly popular variant were the Japanese waltzing mice, so-called because of an inner ear mutation that causes them to circle and spin as they scurry around.1 In the mid-1800s, when Japan opened to the world, British traders described the import of Japanese fancy mice varieties to Europe. Mouse fancying subsequently became popular in Victorian England, where the first national mouse club was established in 1895. Similar to the more familiar dog shows, mice were exhibited and judged against breed standards set by the National Mouse Club.2 An 1898 article describes the show mouse as “larger than the common household specimen. The eye is much larger and fuller, and the coat would not disgrace a thoroughbred racer… the self colours include not only black and white, but almost every shade between.”
Fancy mice may have initially been bred for entertainment, but they were poised to take on a new scientific role upon the rediscovery of Mendel’s laws of inheritance. Mendel first published his findings on the principles governing the inheritance of traits for color and shape in peas in 1866, but his findings languished in obscurity until 1900. Before Mendel, the prevailing theory was that offspring inherited the blended, or “averaged” traits of their parents. It’s likely that Mendel’s discoveries were ignored because they were assumed to be overly narrow. They may explain the color of a flower, but not the more gradually varying polygenic traits like human height that we now know can be affected by hundreds of genes.3
What is striking about the rediscovery of Mendel’s work is that it happened in triplicate; the European botanists Hugo DeVries, Carl Correns, and Erich von Tschermak all performed similar breeding experiments around the same time and, when reviewing the literature, discovered that they had been scooped by Mendel 30 years prior.4 At the time of the rediscovery, few zoologists recognized the fundamental importance of Mendel’s laws as it was not clear that the inheritance of pea flower colors had much to say about animals.
For some open-minded biologists, however, the popularization of Mendel’s work raised questions of whether Mendelian inheritance, with its dominant and recessive traits, applied to animals as well as plants. Mice coat colors were an obvious choice of characteristic to assess at that time, and fanciers were ready to supply a variety of mice. When Mendel began his investigation, his first inclination was to try breeding mice of different colors. However, before his mice experiment really got going, Mendel was forced to switch the subject of his experiments to peas by a prudish bishop uncomfortable with animals having sex in the abbey.

In 1902, Lucien Cuénot was the first to validate Mendelian genetics in mice, when he crossed grey and albino-coated mice and showed that albino coloring was recessive. Cuénot was a zoologist on the periphery of French academia; he worked his whole career in Nancy, never taking an appointment in Paris, even though that was the norm for ambitious scholars in French academic society. Prior to his mouse work, Cuénot had published widely on invertebrate zoology. Cuénot’s reasons for taking up genetic studies of mice have not been fully expounded, but perhaps, isolated from the skepticism of Parisian academic elites who doubted the applicability of Mendel’s laws to animals, he was simply free to follow his own interests.
Secluded in Nancy, Cuénot replicated Mendelian inheritance with other coat color crossings, although some varieties, most notably the yellow-coats, didn’t behave as expected. The interpretation of this anomaly fell to William Castle and his student C.C. Little, researchers at Harvard’s Bussey Institute, who picked up Cuénot’s research and replicated it in 1903. In 1910, the two researchers would solve Cuénot’s yellow mice puzzle by showing that Cuénot had inadvertently discovered a lethal gene. The reason why there were no homozygous yellow mice, in other words, was because they withered away unseen in the womb.
While Cuénot was perhaps the first to operationalize mice for genetics research, his research was cut short by the German invasion of Nancy in 1914. With the outbreak of war in Europe, the Americans took over the reins on turning the mouse into a research tool.
Seeking mice for his experiments, Castle partnered with Abbie Lathrop, a mouse fancier who bred mice on her farm in Granby, Massachusetts. A focus of Castle’s research at the time was establishing how widely Mendel’s laws applied, for which he needed a steady supply of mice varieties (though he also experimented with guinea pigs and rats). While Lathrop had intended to breed her mice to sell to the local fancier community, she inadvertently found herself the supplier for a number of research laboratories, including the Bussey Institute.
Abbie Lathrop was unafraid of rats and mice, character traits that made her something of an eccentric. Or, as an 1898 article in the Reading Eagle about the spread of mouse fancying from Britain to America puts it: “The latest addition to the ranks of pet animals is the mouse, and, strange to say, many women are among those who are interested in it.”
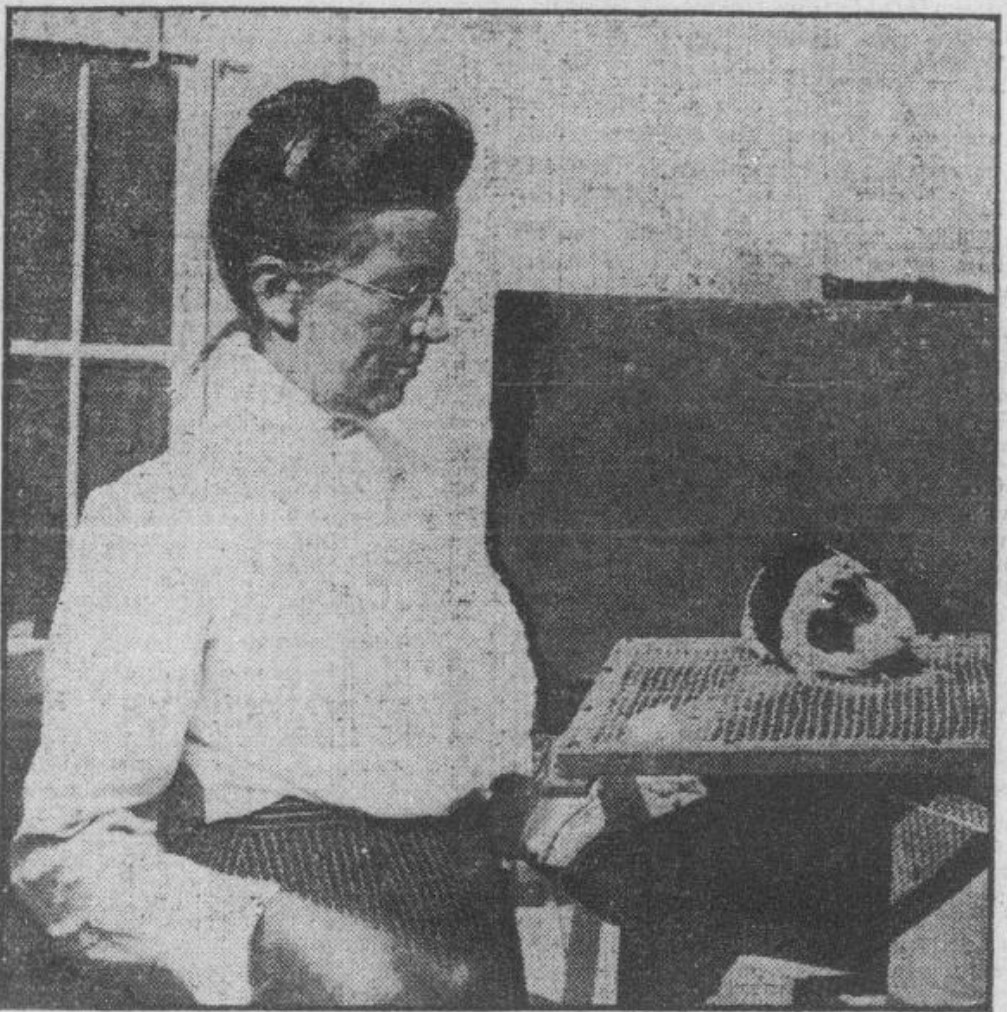
Lathrop’s farm was a mouse melting pot, home to mice from local farmers, as well as more exotic varieties imported from abroad, including Japan. While she had started as a small-time supplier to hobbyists using the offspring of a single pair of waltzing mice, her operation eventually scaled in size and sophistication, with 10,000 mice kept in organized arrays of cages and detailed record keeping on her mice and breeding in the manner of a scientist.5 It was through these keen observations that Lathrop noticed that certain types of mice had a tendency to develop skin lesions.
Eager for an explanation, Lathrop sent her observations to various cancer researchers. Leo Loeb from the University of Pennsylvania responded with a confirmation of the presence of cancer. Subsequently, Loeb and Lathrop began a collaboration that led them to discover that rates of cancer differed between strains of mice, and that cancer susceptibility could be inherited—suggesting a potential genetic basis. Around this time, in 1909, Ernest Tyzzer, another Harvard researcher, was experimenting with transplanting mammary tumors into mice. Tyzzer further advanced the notion of a genetic basis for cancer when he realized that, owing to their relative genetic uniformity, waltzing mice are susceptible to tumor transplantation from one waltzing mouse to another.
While mice were initially used to validate Mendelian genetics, insights into cancer susceptibility suggested that mice could have a more profound role—helping scientists untangle the cause and treatment of human diseases. In a 1913 article titled “The Relation of Heredity to Cancer in Man and Animals,” Little outlined his reasons for viewing the mouse as a model of human disease. While acknowledging the skepticism some expressed in the applicability of mouse data to human disease, he appealed to an argument by analogy: if traits like eye and hair color are inherited in humans as in mice, why should cancer be different? At the smallest scales of the cell and gene, Little argued, mice are similar to men—“it appears certain that the etiology of cancer is a problem of growth and differentiation and as such is essentially biological in nature.”
Although exciting for their potential as a model system, transplanted tumors were challenging to use experimentally. Mice would often reject the implanted tumor, and it was difficult to predict when this would happen. Little reasoned that the variation in susceptibility was driven by a variation in underlying genetics. If he could make less genetically variable mice, he could make them a more useful tool for studying disease.
Little believed that inbreeding mice was the path to creating predictable and genetically standardized mouse models. He was influenced in this line of thinking by another strain of research performed by the Danish botanist, Wilhelm Johanssen. Johanssen created inbred lines of beans by self-fertilizing them for many generations until he had created genetically pure lines.6 Then, Johanssen showed that selective breeding in his inbred beans—selectively choosing and growing larger seeds—had no effect on seed size. Because his beans were genetically uniform, seed size was determined solely by the environment; there was no genetic variation in the population for selection to act upon.
In his 1909 publication on the findings, Johanssen coined the terms “genotype” and “phenotype,” having demonstrated that the outwardly observable traits of an organism can vary for reasons other than genetics. For Little, Johanssen’s research was a demonstration that inbred lines could be genetically stabilized, as if frozen in amber, and thus become resistant to selection effects. This would make them ideal, it seemed, for scientific experiments that required a consistent and reproducible model organism.
Buoyed by Johanssen’s findings, Little performed the first breeding programs to create an inbred mouse strain in 1909. Little’s mentor, Dr. Castle, was initially skeptical. He believed that inbred mouse strains would weaken and become sickly after several generations, losing viability. For what it was worth, Castle was also skeptical that inbred lines could ever reach the desired level of genetic purity, writing: “The biologists’ ‘pure line’ is an imaginary thing, [bearing] no more relation to animals and plants than a mathematical circle has to the circles described by the most accomplished draftsman. All the circles of the draftsman have wiggles in them, if you look at them carefully enough; only the mathematician's imaginary circle is perfect.”
While many of Little’s attempts did indeed fail to create stable lines, he eventually succeeded with a strain he called “DBA,”—for dilute, brown, agouti—in reference to the silvery-brown color of its fur. DBA was quite likely derived from Abbie Lathrop’s partially inbred silver fawn mice. Not only did Little succeed in creating a stable line, but his inbred mice strains also more predictably accepted cancer transplantation. And beyond this, they paved the way to important discoveries, such as the major histocompatibility complex (MHC): the proteins on our cells that signal self-similarity and prevent the immune system from attacking its host.
Bolstered by the success of DBA, Little would later create additional inbred strains at the Bussey Institute, including the C57/B6 strain—or “black 6” to insiders, which is the most frequently used mouse model worldwide.7 Here again, the black 6 precursor can be traced back to mice from Abbie Lathrop’s farm in Massachusetts.
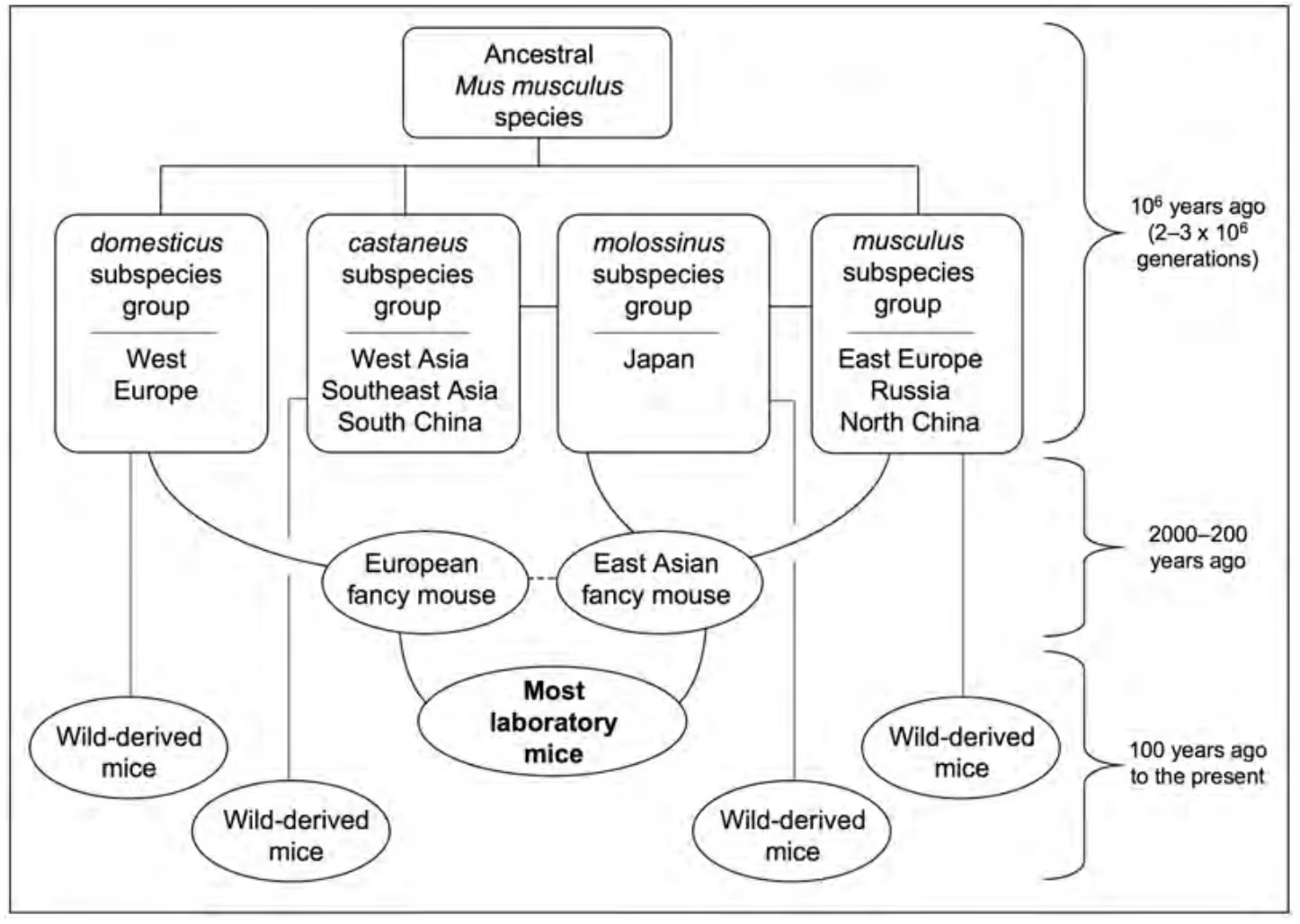
Little moved from Harvard to Maine to take up the presidency of the University of Maine in 1922, and he took his precious inbred mice strains with him. In 1925, Little accepted an offer to become the president of the University of Michigan. Still, he remained focused on his research goals, which were to increase:
“The ‘recognition and appreciation’ of mouse work among physicians in order to ensure ‘the adoption by medical men in general . . . of a biological point of view . . . which will aid immensely in reducing to an analyzable and predictable condition data that would otherwise remain unexplained.”
However, Little felt constrained by the resources available to him within the confines of the academic system. Mice colonies were expensive to maintain at the scale Little desired when compared to simpler model organisms like Drosophila flies; full-time caregivers were needed to maintain the stocks, as well as plenty of space, food, and water. Hygiene standards need to be consistently maintained; an outbreak of disease could at any point demolish the colony and set research back years. To scale up his mouse research and expand his lab in Michigan, Little managed to secure funds from wealthy industrialists, such as John D. Rockefeller. However, even with the mouse research finally garnering the support he felt it deserved, Little’s life in Michigan fell apart. He divorced his wife at the same time disagreements with the University of Michigan administration led him to submit his resignation. Not long after, he returned to Maine.8
Little was ready to begin a new enterprise, and he envisaged a research institute, or “biological resort,” dedicated to advancing the use of the mouse as a tool; one that existed outside of the normal university and patronage systems. As luck would have it, George Dorr, a preservationist known as the “father of Acadia National Park,” was willing to carve out a plot of land near Bar Harbor, and, in 1929, Little founded the Jackson Laboratory there along with seven colleagues.
The goal of the Jackson lab, or JAX, as it’s called now, was to use inbred mice to make fundamental discoveries in cancer, disease, and genetics. Little’s brother, an architect, designed the first building for a minimal budget—a few small rooms equipped with wooden mice cages in unsanitary conditions. Little also had an interest in the genetics of polydactyl cats, which he permitted to roam around the labs and catch stray mice.
JAX was initially supported by a handful of “wealthy Detroiters,” but its patrons withdrew funding as economic conditions soured during the Great Depression. Consequently, the Jackson lab transitioned from a research organization to a commercial one. While JAX had previously given away its mice for free, it was now forced to sell them to make ends meet—selling mice became the lab's sole source of funding. Each mouse cost 10 cents plus delivery, regardless of strain (with an extra charge for specific sexes). Demand quickly outpaced supply, and JAX was soon making enough money to sustain operations. However, the original research mission was a casualty of this pivot, as the top priority of JAX became the mass production of standardized mice for sale.
While the use of inbred mice was initially constrained to research on the genetics of implanted tumors, the research applications of the lab mouse expanded greatly following a 1938 grant from the newly established National Cancer Institute. However, even outside of cancer, policymakers saw the potential of the standardized mouse as a model for studying all manner of human diseases. In a testimony in front of the U.S. Senate and the House on the value of his mice, Little said:
“We can produce as nearly a chemically pure animal . . . as it is possible to produce. . . during this past year the little laboratory where I work has sent out over 65,000 such animals . . . for research in cancer and in other experimental medicine.”9
Little saw the inbred mouse as a standardized reagent that would help drive biomedical progress forward, much like how standardized parts and chemicals enabled the growth of industry. For Little, the use of his mice had always gone beyond cancer; in his speech to Congress to advocate for funding the JAX, Little painted a vision of a world in which, by understanding the mouse, we would come to understand ourselves.
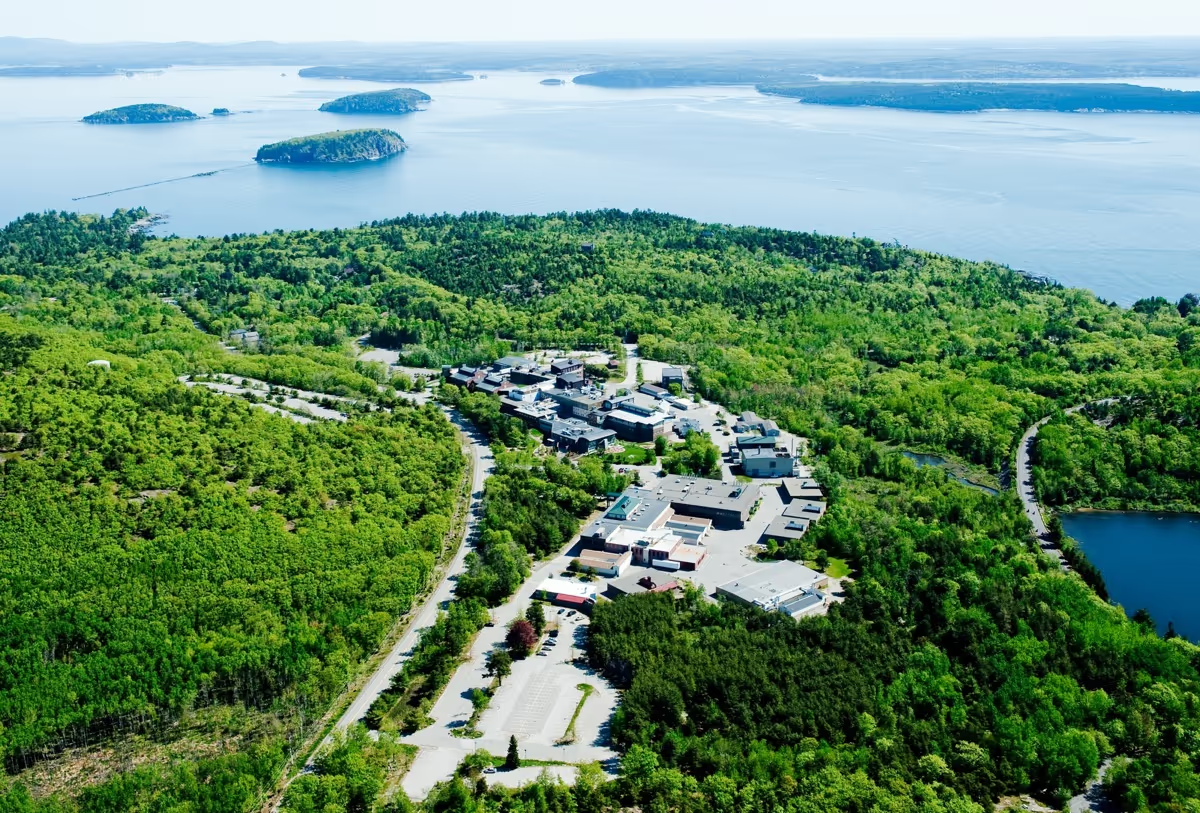
Inside the Mouse Monastery
If there is anything like a modern monastery, or temple, devoted to biomedicine, The Jackson Laboratory is it. The nonprofit organization is situated in dense pine forests on the eastern coast of Mount Desert Island, a glacier-scarred land torn in half by the Somes Sound fjard, near Acadia National Park. JAX buildings look over the water and the small islands that, like stepping-stones, sit between Bar Harbor and the scraggly coast that marks the northeastern terminus of the United States.
Inside its several dozen blocky teal and sandstone buildings, JAX maintains 13,000 strains of mice and ships millions of mice to researchers per year. As our control over biology has expanded, so has JAX’s catalog. Researchers are no longer limited solely to strains bred manually or spontaneously discovered, but can now use tools like CRISPR to knock in or out specific genes to create bespoke mice to model disease.10 While JAX is no longer the only large vendor of laboratory mice—Charles River Laboratories, founded in 1947, is the world’s largest commercial mouse distributor—today’s most popular strains can nevertheless trace their origin back to JAX. Charles River’s best seller is a variety of black 6 (C57BL/6NCrl) that found its way to the company through JAX and the NIH.
If JAX is a temple, then colony management is the ritual. The JAX handbook of genetically standardized mice details the procedures to keep the colony free from microbial contamination, from physical barriers to procedures for how to enter and travel through the mouse rooms. When new mice are introduced from the outside world, extreme measures are taken to prevent contamination; pregnant female mice are killed, their uteruses surgically removed and passed through a disinfecting barrier before the live young are extracted and given to a new “pathogen-free” mother.
Just as important, if not more important, than maintaining a barrier with the outside world are the procedures that maintain the genetic purity of the prized JAX inbred lines—the Genetic Stability Program.
While some contamination of the gene pool is a result of inadvertent crossings between strains, there is also the inexorable genetic drift: the spontaneous occurrence and fixation of novel genetic mutations. To prevent genetic drift, the most popular strains—like black 6—are bred from foundation stocks which are regularly genotyped with a panel that looks at 27 single nucleotide polymorphisms (SNPs). Mice stocks (ova, embryo) are cryopreserved on campus and at a backup location, in case of a disaster that would threaten the facility. And every five years, the foundation stock is reestablished from frozen embryos:
“The result is that, within a 100-year period, a foundation stock will advance no more than 4–10 generations. This is in contrast to even the best colony management production practices, which result in about 3 generations per year—about 300 generations over a 100-year period. This effectively retards the rate of genetic drift by 20- to 50-fold.”
While this slows genetic drift substantially, it doesn’t eliminate it. More than 40 substrains of black 6 arose between 1930 and 1970 as a result of genetic drift, with subtle differences in physiology. Materials from the JAX lab warn about the impact that substrain differences can have on research conclusions. In one case, a gene that appeared to be toxic to the liver when using one substrain of black 6 as a control seemed to instead protect liver function when a different substrain was used. These differences cost researchers time and money to chase down and, likely, often go unnoticed. Even slight differences between substrains can lead to large differences in the disease models generated with these strains, which affect how they respond to antiepileptic drugs, implanted tumor rejection, insulin secretion, and more.

Although variation in the mouse stock is not created intentionally, it often proves useful. In such a large colony, there are bound to be spontaneous mutations with interesting phenotypes. To find these, JAX trains its technicians to look out for “deviants”; any mice with unusual characteristics not typical of the parent strain are set aside in case they may be useful for researchers. In one such occurrence, Leroy Stevens—at the time, a graduate student working at JAX—found a teratoma (a nightmarish tumor of hair, muscle, teeth, and bone like a malformed fetus) in one of the mice. There had never been a model for these malformations, and after investigating further, Stevens found that one percent of mice of a certain strain harbored teratomas. With further searching and genetic manipulation, Stevens was able to reliably produce teratomas in mice. Subsequently, Stevens noticed that the cells that spawned teratomas were similar to cells found in embryos. Later scientists built on Stevens’s observation to show that “embryonic stem cells” are capable of developing into any cell in the body, and so may one day be useful tools for replacing damaged or diseased tissues more broadly.
It’s ironic that we have learned so much from variation even as biomedicine has tended towards ever greater standardization. Black 6 is the most popular strain of mice used in research today, but its status as a standard is due to little more than chance. Because it became popular early in the history of mouse fancying, a great body of research and methods were published using the black 6 mouse, which locked in its continued use. Yet the black 6 mouse is unusual in many ways: it’s sensitive to cold and pain, becomes easily addicted to alcohol and morphine, and is prone to biting. Black 6 may seem normal because it’s ubiquitous, but that’s only because standardization has locked in its idiosyncrasies. Even for those aware of these issues, there may be little they can do to escape the pull of the standard.
Biomedical sciences have been criticized as “an internally self-consistent universe with little contact with medical reality.” It’s an uncharitable take, but when drugs fail as often as they do despite animal testing, it’s not hard to see the JAX mice as a metaphor for a biomedical enterprise cloistered away from the real world. We may be spending a great deal of time and money on work that has the illusion of fruitfulness, and there are good reasons to believe that inbred mice often lead us astray.
For one, the high apparent degree of genetic similarity between mice and humans may be misleading. While the protein-coding regions of the mouse genome are similar to those in humans, there are substantial differences in the sequence of non-coding regions. The chromosomes of the rodent underwent substantial shuffling after we diverged from our common ancestor, leading to macrostructural differences—even though we share many genes, they’re in different places.
While fundamental research is often productive in mice, translational drug research tends to be relatively intolerant to biological differences. In cancer, the focus of Little’s ambitions, mouse models leave much to be desired. Implanted tumors (including xenografts) are now a standard part of preclinical cancer drug research, yet our success rates in cancer drug development are among the lowest of all therapeutic areas. Compared to natural humor tumors, implanted tumors tend to be more genetically uniform, as well as having different (often simpler) architectures and microenvironments, which complicates the transferability of results.
Even diseases that are genetically simple can be hard, or impossible, to recreate in mice: deadly diseases like Tay Sachs and Duchenne muscular dystrophy (DMD) are caused by mutations in single genes in humans, but knocking out the relevant genes in mice leads to much milder symptoms. While mouse models of these genes have provided insight into the basic biology, there are substantial differences that impede therapeutic translation. Some of this is due to size: we are much bigger than mice, and the biophysical stressors on our bodies lead to differences in how the effects of genes manifest with scale. In other cases, mice may have different compensatory mechanisms from ours.
This raises a question: what is the purpose of biomedical science? Because if it’s to efficiently discover new drugs, our investment in the enterprise of mouse models leaves much to be desired. Little’s vision of using mice as a stand-in for humans was never fully realized; when it comes down to the details, we may be too different. Alternatively, if the purpose is to pursue curiosity-driven research in the hope that mouse discoveries translate to humans, we might inadvertently be spending all our resources following a narrow alleyway with nothing at the end. After all, the in-depth study of any one organism is a kind of Socratic trap where no matter how many questions we ask—even if we focus on specific models and strains—more questions arise, each of which takes resources to answer.
Since—when it comes to therapeutics, at least—we ultimately care most about furthering our understanding of human biology, it seems likely that we’d benefit from refocusing some attention towards accelerating the start of human testing. For instance, FDA guidance allows for “phase 0” trials, which allow tiny doses of investigational drugs to be tested in humans, without extensive prior preclinical work, to understand how these new drugs are metabolized and engage targets in the body. The FDA itself believes these types of early human studies are underutilized.
But there also seems to be a great deal of untapped opportunity in non-rodent models. The biosphere has an abundant collection of potential new models. The oft-unrealized ideal in research is to “select the best model for the job,” but in practice, researchers default to the familiar black 6 for reasons of feasibility or familiarity. Some are working to change this: for instance, Fauna bio is collecting genomic data from a wide variety of animals, including fringe critters like the 13-lined ground squirrel, that might help us better understand highly conserved genes and resistance mechanisms to diseases like obesity. Now that the cost of sequencing is coming down, this type of data collection is more feasible than it once was.
It’s plausible we should even abandon the use of animals entirely and focus on human organs-on-chips, mini-organs, or AI models. This might benefit not only science but also the millions of animals who would otherwise suffer or die in the course of biomedical research. While there’s little incentive for this infrastructure-building work in academia or industry, a well-resourced focused research organization (FRO) or ARPA-H might be an ideal structure to explore the space of new models.
One of the biggest problems holding back our exploration of new models is a lack of funding, which is downstream of an entrenched rodent-centric culture. Most labs are equipped to deal with mice, and each animal has its own set of preferences that need to be catered for (often at great expense). Industry and grant funders are often “mouse people,” and aren’t sure what to make of proposed studies in unusual models. Even though the NIH has a group that funds the development of new models, their budget is relatively small. While these groups may provide initial start-up funds, their funding is often not consistent enough to see these projects through to completion: for a case in point, see the recent curtailment of funding for the long-running dog aging project. A greater diversity of funding sources could help; in practice, the NIH’s near-monopoly on biomedical research funding means that if the NIH is not receptive, there’s often nowhere else to turn.
It’s also worth noting that we could make better use of the mouse. Poor quality research, unnoticed substrain variation, and/or flawed model choice, impair the translatability of findings and can make the mouse appear to be a worse model than it actually is. One option is to move towards more natural models, whether that’s pre-aged mice that might be better fits for studying the biology of aging or more genetically diverse mouse strains. The reasoning here is that part of the failure of translation from mouse studies to human studies may not be differences between mice and humans in general, but rather between humans and lab mice specifically. Beyond genetics, differences in feeding, housing, and handling can make a large impact on results; although JAX takes great care in how they manage their mice, the same doesn’t necessarily apply to the downstream labs that use their mice.
The remedy, then, is not to discard the venerable mouse—for it has taught us much and may still have more to teach—but to work harder to explore the variety and diversity of other forms of life. We should once again embrace variation (as the mouse fanciers did) and perhaps ask more questions of other organisms and fewer of the mouse.
{{divider}}
Alex Telford is the founder of Convoke, a technology company that helps biopharma companies make better development and commercialization decisions, and a former consultant to the industry. In his spare time he enjoys blogging about biotechnology.
Note: Much of this essay draws from details published in the book “Making Mice” by Karen Rader.
Cite this essay: Alex Telford. “The Mouse as a Microscope.” Asimov Press (2024). DOI: doi.org/10.62211/75pw-88uu
Lead image: Waltzing mice by Charles Hermann-Léon, 1891.
Footnotes
- These mice were probably Chinese in origin but acquired the “Japanese” descriptor because of a miscommunication between a Japanese mouse dealer and the European who acquired the mouse from them.
- A special type of cage called the ‘Maxey cage’ was invented to display mice for shows. These cages are still used today.
- How Darwin’s theory of evolution and natural selection could be reconciled with Mendelian genetics was a significant point of dispute in the early 20th century. Francis Galton showed that human children tended towards the average of their parents on many traits, such as height, which suggested that inheritance was blended. However, if blended inheritance were true, beneficial traits would likely be averaged out before selection had a chance to act. The tension was resolved with the ‘Modern synthesis,’ which showed the compatibility of the two paradigms of Darwinian evolution and Mendelian genetics. We now know that continuous trait values are the result of the influence of many genes.
- William Jasper Spillman, in the U.S., also rediscovered Mendel’s laws and published in 1901.
- “Making Mice,” by Karen Rader.
- Technically, homozygous at every genetic locus.
- The reasoning is somewhat circular, but it is often suggested that the black 6 mouse is popular because it is so widely used. This creates a cycle wherein researchers then use it subsequently to reproduce results. Other articles cite its relative robustness and ease of use and breeding as justification, but these properties are not unique to black 6.
- In “Making Mice,” Rader suggests that Little was “not a man to back away from a ‘fighting attitude’ on any issue in which he thought he was right.”
- “Making Mice,” by Karen Rader.
- Mice are especially useful in part because they are relatively amenable to alteration; mouse embryonic stem cells are unusually easy to grow (compared to other species, even closely related ones like rats). This allowed researchers to easily engineer mice by editing the stem cells, and then injecting the edited cells into a mouse embryo. This method was developed in the 1980s, yet similar methods were not developed in rats until the 2010s, and are still unavailable for most species.
This article was published on June 2, 2024.
Always free. No ads. Richly storied.
Always free. No ads. Richly storied.
Always free. No ads. Richly storied.