
Intelligence means perceiving information and then applying it to adapt to different contexts. That sounds an awful lot like what cells do.
Consider the humble Escherichia coli, which uses its whip-like flagella to swim. In an article titled, “The Baffling Intelligence of a Single Cell,” writer and programmer James Somers describes how these microbes chase their food by swimming up a gradient “using purely biochemical cues.” This feat is called chemotaxis, a word derived from “chemo-” for chemical and “taxis” from the Greek τάξις; or, as Somers writes, “intelligence in one of its most elemental forms.”
A recent paper in the journal Science pushes the notion of cellular intelligence further still. In just six wonderfully lucid pages, researchers from Vanderbilt University in Nashville show that cyanobacteria can “sense” shortening days and change the molecular compositions of their cell membranes to prepare for cold weather.
This discovery would be entirely unremarkable in mammals; we already know of many examples of animals that hibernate, migrate, or estivate in response to seasonal changes. But this study documents the first time that “photoperiod adaptations” have been observed in a single-celled organism. Cyanobacteria — an organism that divides in just six hours — is able not only to sense changes in the hours of daylight but also to use that information to adapt and prepare for weather far in advance.
The intelligence of cells extends even further than we thought, and a molecular clock lies at the heart of it all.
{{signup}}
Clocks in Nature
Circadian clocks appear nearly everywhere in nature.
Male canaries sing more in the spring because, as the days lengthen, their testes grow in size, producing more androgen hormones that induce mating behaviors. Plants use pigments to track the length of nights and control their flowering patterns, a phenomenon first documented in the 1800s. Fruit flies emerge from their cocoons around dawn, when the air is damp, allowing their wings to expand without drying and becoming too brittle. The human circadian clock ticks in roughly 24-hour periods; mutations in the underlying genes can disrupt sleep rhythms, dictating whether someone is likely to be an early or late riser. These phenomena have been well-documented for more than a century.
Prokaryotes, however, were not known to possess their own “rhythms” until the late 1980s, when two biologists at the University of California, Santa Barbara — named Beatrice Sweeney and M. Beatriz Borgese — grew Synechococcus cells (ancient cyanobacteria fished from the ocean waters near Bermuda) in their laboratory.
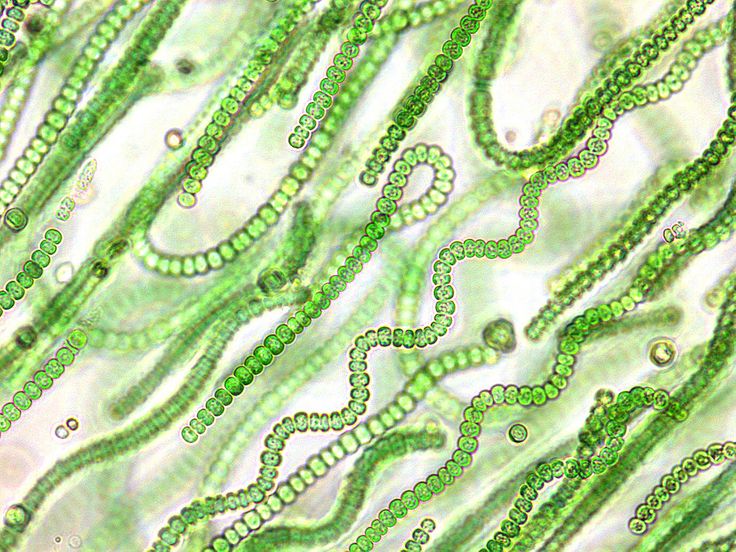
Sweeney and Borgese grew the cyanobacteria in a room with alternating patterns of light and darkness — 12 hours each. They consistently saw “peaks in cell division … in the first part of the night … irrespective of the Pacific Standard Time of the light and dark periods.” In other words, cyanobacteria were more likely to divide toward the start of the dark cycles, regardless of what time it was outside the laboratory. This periodicity was maintained over several days, “like clockwork.”
Direct biochemical evidence of a cyanobacterial “clock” followed in 1993. A group of Japanese and American researchers plucked a gene encoding luciferase, an enzyme that performs a chemical reaction to produce light, from a bioluminescent microbe called Vibrio harveyi. Then, they pasted the luciferase gene into a cyanobacterium, just downstream of a gene called psbAI, which encodes an important protein involved in photosynthesis.
These scientists hypothesized that the circadian rhythms of cyanobacteria might somehow be linked to photosynthesis, perhaps an unsurprising idea given that these primordial microbes first evolved enzymes to pump oxygen into Earth’s atmosphere more than 2.5 billion years ago. If they were correct, then they should be able to “see” the cells cyclically ramp photosynthesis up and down by measuring the amount of light they produce via luciferase.
That’s precisely what occurred. The genetically modified cyanobacteria were grown in a 12-hour light and 12-hour dark pattern, and their luciferase levels went up-up-up and then down-down-down in a roughly 24-hour period. When the light patterns were adjusted, the cyanobacteria adjusted their rhythms accordingly. The experiment, at last, offered biochemical proof that prokaryotes have circadian rhythms much like birds and humans.

What were these clocks made of, the scientists wondered? Anyone who has seen a metabolic network diagram knows that biology is complex. One would be forgiven for assuming that cyanobacterial clocks would be composed of dozens of interacting proteins. But instead, the clocks’ mechanisms turned out to be surprisingly simple, even though it took a cleverly designed experiment to reveal it.
In 1998, a Japanese group discovered the causative genes by using transposons, or small DNA sequences that insert themselves into genes, to randomly disrupt genetic functions inside cyanobacteria cells. After mutating and isolating tens of thousands of cyanobacterial cells, they searched for those with altered circadian rhythms — too fast, too slow, or depleted entirely — and found just 19 mutants. In each of those mutants, disruptions to just three genes caused the circadian rhythms to go haywire. The Japanese scientists named the genes kai, which means “to change” in Japanese, and labeled them kaiA, kaiB, and kaiC.
Swatch, the Swiss watchmaking company, once made a mechanical watch movement from just fifty-one parts, a remarkable feat considering that about 180 parts comprise a typical mechanical watch. They named their creation the Swatch Sistem51. That nature managed to evolve something similar, with more than an order-of-magnitude fewer parts, astonishes.
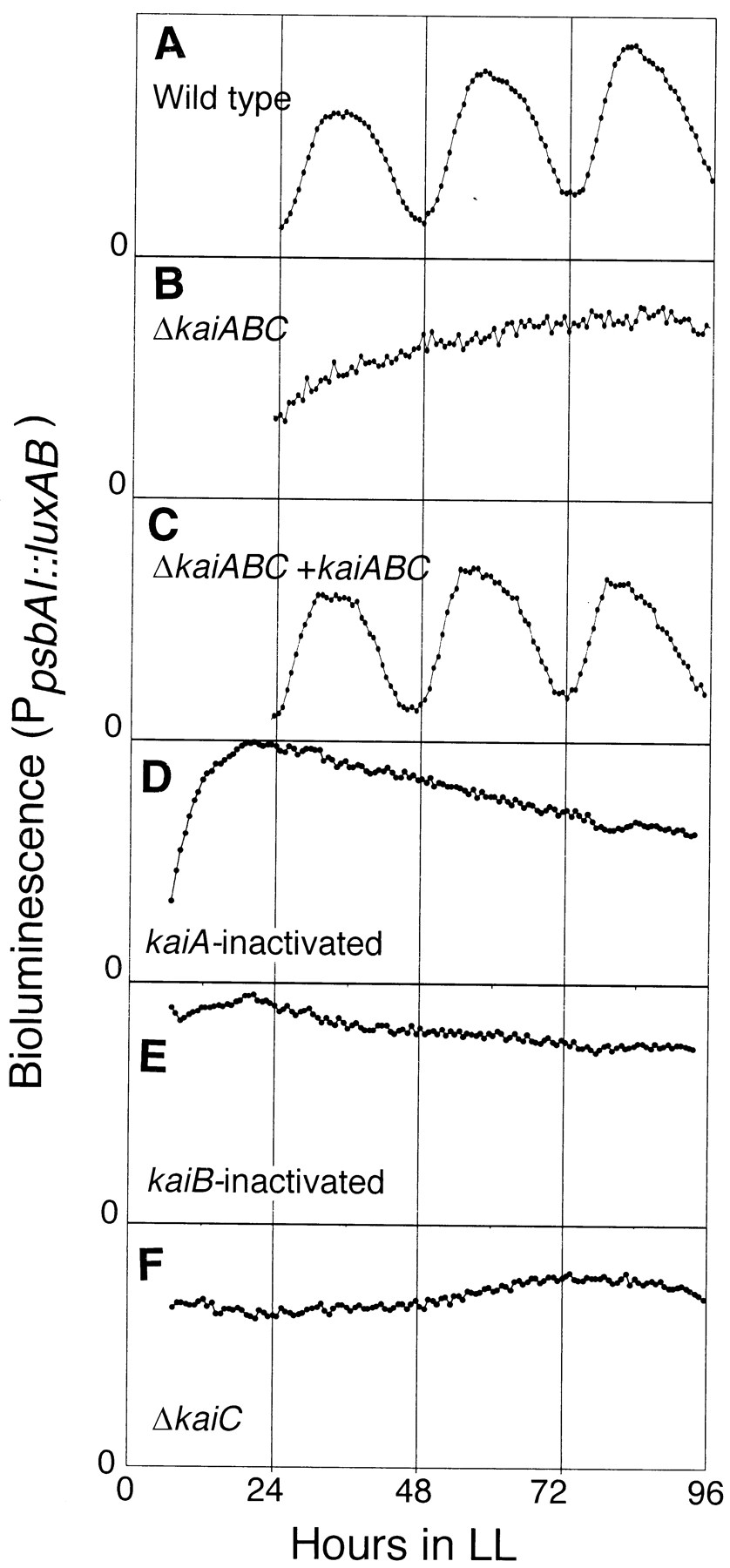
KaiC forms the core of the clock. Specifically, six KaiC proteins link together to form a ring. The clock starts ticking when KaiA binds to this ring, inducing KaiC to begin adding phosphate molecules onto its own amino acids. These phosphates are first stuck onto threonines, then onto serines, until all of the phosphate “slots” fill up.
The clock starts to wind down when KaiB binds to KaiC, pushing it into a new position. As KaiC shifts, a binding site opens up on KaiB that tightly binds to KaiA molecules, sequestering them. As KaiA proteins are sequestered away, KaiC stops adding phosphates onto itself and instead begins to throw them off. Once all the phosphates have fallen off (the two phases take almost exactly 24 hours), the clock resets. KaiB falls away from KaiC, releasing the sequestered KaiA molecules. The cyanobacterial day begins anew.
This clock runs autonomously. It is powered, much like chemotaxis, by purely biochemical signals. The kai clock is so impressive, in fact, that it will continue to oscillate with nearly perfect 24-hour periods even when the three proteins are plucked from their microbial hosts and placed into a test tube. This is akin to a deconstructed mechanical watch, lacking gears and springs, tracking time purely through the transfer of atoms between its cogs.
Changing with the Seasons
A few years ago, a graduate student named Maria Luísa Jabbur approached her thesis advisor, Carl Johnson, and asked if she could test a hunch: that cyanobacteria might use their kai clocks to not only track the time but to anticipate the seasons.
Carl Johnson was just the person to ask about this intuition because he was the first to discover that cyanobacteria have circadian rhythms way back in the 1980s. He also worked with the Japanese group to describe the kai genes in 1998. Even so, he found Jabbur’s idea dubious.
Still, Jabbur told a university press writer: “he told me to go away and try it, and as I went, he showed me a sign on his door with the Frank Westheimer quote: ‘Progress is made by young scientists who carry out experiments that old scientists say would not work.’”
The experiment worked the very first time.
It happened like this: Cyanobacterial cells were divided into three groups. Each group grew at the same temperature — a steady 30°C. But each group was exposed to a different amount of light each day. One group was exposed to 16 hours of lightness and 8 hours of darkness each day; another to 12 hours of light and 12 hours of darkness, or “equinox”; and the third to 8 hours of light and 16 hours of dark.
After eight days, Jabbur dunked each group of cells into ice-cold water and measured how many lived through the ordeal. Cells exposed to less light (8 hours light, 16 hours dark) were two-to three-times more likely to survive compared to the other two groups. The effect was also linearly correlated. Cells exposed to 20 hours of darkness per day were more likely to survive the cold water compared to cells exposed to 18 hours, and so on.

This effect went away entirely, though, when any of the kai clock genes were deleted or mutated, suggesting that cyanobacterial rhythms are somehow involved in sensing shortening days as winter approaches and then equipping the organism to survive in colder weather. How this happens, though, wasn’t immediately clear.
Again, a prior paper pointed the way forward. In 2011, Chinese researchers found that cyanobacteria growing at 30°C quickly die off if they are dipped in 5°C water. But if the researchers first exposed cyanobacteria to 15°C, the cells somehow “adapted” and didn’t die off in the 5°C water. The researchers discovered that cells “trained” in the 15°C water shifted the molecular composition of their cell membranes to prepare for future cold plunges.
The cell membrane, for context, is made from wiggly fat molecules called lipids. When hydrogen atoms are stripped from lipids — a process known as desaturation — double bonds form between carbons and the lipid becomes kinked. These kinks prevent lipids from packing too closely together and thus make the cell’s membrane both more flexible and more able to withstand frigid temperatures. Perhaps, Jabbur postulated, the kai clock desaturates lipids in a similar way.
Jabbur repeated the experiments, but killed all the cells and isolated their lipids for further study at the end. Cells exposed to more darkness each day had more desaturated lipids, and cells with mutated kai genes that were exposed to the same amount of darkness had no changes in their lipids. Adaptation to cold weather, in other words, depends upon the kai clock.
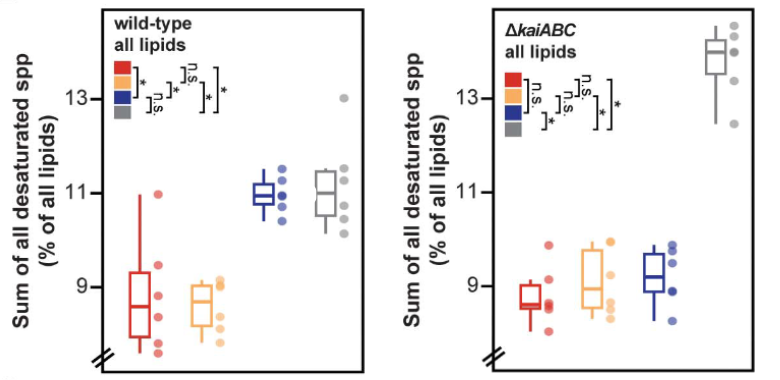
But unfortunately, this is where the paper starts to get fuzzy. The authors never managed to directly tie kai genes to cyanobacterial proteins that rewire the cell membrane. All we know for sure is that cells exposed to less light each day had more than 700 differentially-expressed genes compared to cells exposed to more light. Many of these genes are involved in fatty acid metabolism and the cell cycle. There is no way to say, definitively, that this gene or that gene connects the kai clock to the cell membrane; such an assertion must wait for a future paper.
Despite being unable to verify a clear-cut mechanism, this paper is profoundly beautiful. Photoperiod adaptations have long been observed in multicellular organisms and eukaryotes, but this study suggests this capability may even predate the evolutionary emergence of multicellular organisms 2.7 billion years ago as — based on fossils discovered in rocks from Western Australia — cyanobacteria first appeared an estimated 3.5 billion years ago. This gives us a different picture of single-celled life on our primordial planet. Even before the dawn of eukaryotes, organisms sensed their environment and adjusted accordingly.
Most importantly, this paper provides further evidence that bacterial cells — which seem simple by our multicellular standards — have likely survived on this planet for so long because they are far more intelligent than we give them credit for.
***
Niko McCarty is a founder of Asimov Press.
Cite: McCarty, Niko. “How A Cell Anticipates the Seasons.” Asimov Press (2024). DOI: https://doi.org/10.62211/91tt-45jk
Always free. No ads. Richly storied.
Always free. No ads. Richly storied.